Simulation of Atomic Force Microscope Stretching Experiments I - Mechanical Properties of Polysaccharides
Berthold Heymann and Helmut Grubmüller
Collaborators: Filipp Oesterhelt, Hermann Gaub (LMU Munich), Matthias Rief (TU Munich), Hongbin Li (Rochester, USA).
Support: DFG (SFB 143/C1)
Elastic properties of three biologically relevant D-glucose polymers (1-6)-glucose (dextran), α(1-4)-glucose (amylose), and β(1-4)-glucose (cellulose) have been studied by molecular dynamics (MD) simulations [1,2]. In these simulations, the sugar polymers were stretched by an external force in close resemblence to recent AFM experiments in Hermann Gaubs lab in Munich.
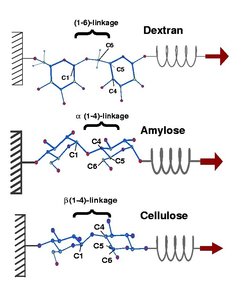
The three sugar polymers dextran (top), amylose (middle) and cellulose (bottom) consist all of the same glucose monomers. They differ solely in their monomer linkages. Wheras in dextran the monomers are connected via the carbon atoms C1 and C6 [(1-6)-linkage], in amylose and cellulose the monomers are linked via the carbon atoms C1 and C4 [(1-4)-linkage]. Amylose and cellulose, in turn, slightly differ from each other in the sterical arrangement of the linkage: As visible in Figure 1, the linkage is pointing downwards in amylose (middle) and upwards in cellulose (bottom).
In the stretching simulations one end of the polymer was fixed, whereas the other end was attached to a harmonic potential (in the figures symbolized by a spring) which closely models the cantilever of an atomic force microscope. In the course of the simulation, the spring was moved towards the right (red arrow) with constant pulling velocity, thereby stretching the polymer. The stretching force was recroded as a function of the polymer extension, resulting in a force extension curve (see Figure 2), which reflects the elasticity of the polymer. The simulations where carried out with polymers of different lengths up to 16 monomers (for clarity only dimers are shown in the Figures). Since a couple of control simulations revealed no significant effects of solvent, especially not on the elastic properties, most of the simulations were carried out in vacuo.
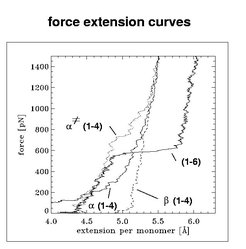
As visible from Figure 2, all force extension curves significantly differ from each other. For dextran [line denoted as '(1-6)'], three regimes can be distinguished: a first regime of mean elasticity (mean slope of the curve), a second regime of high elasticity at ca. 600 pN (pronounced plataeu of the curve), and a third regime of low elasticity. The first regime was already known from experiments, the second and thrid regime were predicted by our simulations. These could be experimentally reproduced afterwards, as it had become possible to apply forces up to 800 pN to single polymer molecules.
In contrast to dextran, (1-4)-glucose ['(1-4)'] shows a continous stiffening in its force extension curve. However, restricting the intermonomeric rotational flexibility [curve denoted as '(1-4)'], which models α(1-4)-glucose used in the AFM experiments, the elastic properties significantly change, resulting a plateau in the force extension curve, which is, however, less pronounced as in dextran. In contrast to the other suger polymers, β(1-4)-glucose (dashed line) does not exhibit significant changes in its elastic properties upon stretching forces above 100 pN. The elasticities derived from these curves agreed well with the experimental values.
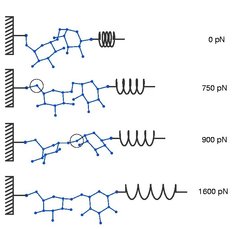
Thus our stretching simulations revealed in close agreement with AFM stretching experiments that very slight differences in the molecular structure have dramatic consequences for the elastic properties. What is the reason for that? How do the molecules behave under mechanical stress?
The MD simulations provided interpretations for the measured elasticities in terms of structural changes induced by the applied mechanical stress.
For α(1-4)-glucose and β(1-4)-glucose, our simulations revealed also conformational changes, however different from those in dextran: Whereas the rings in α(1-4)-glucose underwent a transition from 'chair' to 'boat' conformation upon mechanical stress, in β(1-4)-glucose the monomers did not, but instead slightly tilted with respect to each other. The continous stiffening of α(1-4)-glucose with completely preserved intermonomeric rotational flexibility is caused by the fact that the 'chair' to 'boat' transitions do not occur more or less simultaneously, i.e. upon a certain stretching force, but take place over a wide range of stretching forces.

Accordingly, the elongation of α(1-4)-glucose is a rather continuous process. The deeper reason for it is a significant anti-cooperativity of the 'chair' to 'boat' transitions: if one monomer flipped from 'chair' to 'boat', transitions of neighboured monomers require much higher stretching forces. This anti-cooperativity does not occur, if the intermonomeric rotational flexibility is suppressed as is in the case in the AFM experiments due to bulky side groups of the monomers.