One enzyme, two mechanisms: How tRNAs are trimmed in the nucleus and mitochondria
A team led by Hauke Hillen, research group leader at the University Medical Center Göttingen (UMG) and the Max Planck Institute (MPI) for Multidisciplinary Sciences, has now uncovered the molecular mechanism by which the 3’ ends of transfer RNAs (tRNAs) are processed in the nucleus and mitochondria of human cells. The researchers’ findings not only provide an important puzzle piece for the basic understanding of gene expression. They may also help to develop new strategies for treatment of human diseases such as mitochondriopathies caused by defects in mitochondrial tRNA processing.
Proteins are the workhorses of all living organisms, as they carry out almost all tasks required for life. To produce proteins, the blueprints encoded in the genes of the DNA are first copied into RNA in a process called transcription. While DNA and RNA are made up of nucleotides, proteins are built from amino acids. The translators between these two different chemical “languages” are tRNAs. These molecules read the sequence of letters encoded by DNA and translate this information into a sequence of amino acids. The evolutionary ancient tRNAs are thus essential for all life on earth.
When tRNAs are first made in the cell, they contain extra nucleotides at both ends, called 5’ and 3’ ends, which need to be removed in order for the tRNA molecules to become functional. This step, termed tRNA processing, is carried out by enzymes called ribonuclease P and ribonuclease Z (RNase P and RNase Z for short). The tRNA processing typically proceeds via two steps: First, the extra nucleotides at the 5’ end are trimmed away by RNase P and then RNase Z takes over to remove the extra nucleotides at the 3’ end.
tRNAs have a typical L-shaped structure, which is important for their function and is also recognized by many enzymes involved in their biogenesis. This tRNA structure arose very early in evolution and has ever since remained unchanged in all groups of organisms that exist today – with one notable exception: In animal mitochondria, tRNAs have lost many of the typical structural features that are normally required for tRNAs to function. Therefore, in these organelles specialized mitochondrial RNase P and Z enzymes called mt-RNase P and mt-RNase Z ensure that the mitochondrial tRNAs are processed correctly. If these machineries do not function properly, for example due to genetic mutations, this can lead to severe human diseases called mitochondriopathies. However, the precise molecular mechanisms of how mitochondrial tRNAs (mt-tRNAs) are processed and how defects in this process cause disease are not well understood.
Unique mechanism to recognize mt-tRNAs
Hillen’s team has previously succeeded to elucidate how the 5’ ends of mt-tRNAs are processed. The scientists showed that the human mt-RNase P differs dramatically from its counterpart in the nucleus and that it employs a unique mechanism to recognize mt-tRNAs by joining forces and forming a complex with seemingly unrelated enzymes, the methyltransferase TRMT10C and the dehydrogenase SDR5C1, which help to recognize the structurally unusual, “non-caninocal” mt-tRNAs.
In their newest research, the scientists now unraveled the molecular mechanism of RNase Z. In human cells, the 3’ ends of both nuclear and mt-tRNAs are processed by the same RNase Z enzyme – also called ELAC2. Intriguingly, ELAC2 can process nuclear tRNAs all on its own. But for processing of most mt-tRNAs, it requires the same additional proteins also found in mt-RNase P: TRMT10C and SDR5C1. RNase Z thus exists in two forms in human cells: A nuclear version which consists only of ELAC2 and a mitochondrial form in which ELAC2 associates with TRMT10C and SDR5C1 to form mitochondrial RNase Z.
Molecular recognition of canonical nuclear and mt-tRNAs
But why does ELAC2 require additional players only for processing mt-RNAs? To answer this question, the researchers reconstituted tRNA processing in a test tube using highly purified proteins and tRNAs. Their experiments showed that TRMT10C and SDR5C1 proteins are only required for 3’ processing of those mt-tRNAs whose structures are very different from the “canonical” tRNA structure. In contrast, nuclear tRNAs and mt-tRNAs with a canonical structure can be processed by ELAC2 alone.
By systematically altering the sequence and structure of the tRNAs used in their system, the team could pinpoint the exact part of the tRNA that determines whether it can be processed by ELAC2 alone, or whether help of TRMT10C and SDR5C1 is needed. “This part of the tRNA is known as the ‘elbow’,” explains Bernhard Kuhle, one of the authors of the study. “Our analysis showed that this structural feature was either much smaller or even absent in those mt-tRNAs that required additional proteins for 3’ processing. This suggests that ELAC2 recognizes canonical nuclear and mt-tRNAs through their elbow, and that TRMT10C and SDR5C1 compensate for the absence of this feature in non-canonical mt-tRNAs.”
As a next step, the team wanted to find out exactly how TRMT10C and SDR5C1 help ELAC2 to process structurally non-canonical mt-tRNAs. To observe this, the researchers used single-particle cryo-electron microscopy, which allowed them to visualize the molecular structure of the mitochondrial RNase Z complex, consisting of ELAC2, TRMT10C and SDR5C1, at near-atomic resolution. They determined structures of this molecular machine with either a canonical mt-tRNA, which ELAC2 can process alone, or a non-canonical mt-tRNA, for which ELAC2 requires TRMT10C and SDR5C1 (Figure 1).
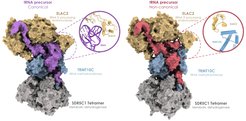
“While the structures appear very similar at first glance, there are striking differences in how ELAC2 interacts with these two tRNAs,” Hillen says. In the case of the canonical tRNA, ELAC2 directly interacts with the tRNA elbow. The non-canonical tRNA, however, does not have an elbow and ELAC2 instead interacts with TRMT10C, which in turns binds the remaining parts of the tRNA together with SDR5C1.
Additional proteins act as molecular prosthesis
“The two accessory proteins TRMT10C and SDR5C1 thus act like a molecular prosthesis, compensating for the loss of protein-RNA interactions with non-canonical tRNAs by establishing unique protein-protein interactions between ELAC2 and TRMT10C,” explains Arjun Bhatta, who carried out the structural analysis. This enables ELAC2 to process structurally unusual tRNAs that lack an elbow, such as those found in human mitochondria.
One question that still remains to be fully answered is why tRNAs in animal mitochondria differ so dramatically from the “canonical” tRNA structure in the first place. A possible reason could be the high mutation rate of the mitochondrial genome, which mutates much faster than the DNA in the cell nucleus. When mutations occur in the mitochondrial tRNAs that affect their structure or function, these could potentially have dramatic consequences for the organism.
The team’s new findings suggest that TRMT10C and SDR5C1 could have evolved as a compensatory mechanism, which enables correct processing despite the lack of canonical structural features in the tRNA. “Indeed, an evolutionary analysis shows that TRMT10C and SDR5C1 first appeared around the same time when mt-tRNAs started to ‘degenerate’ in animals,” says Bhatta. Thus, the “molecular prosthesis” formed by TRMT10C and SDR5C1 appears to be a general mechanism to compensate for the structural heterogeneity of mitochondrial tRNAs.
Taken together, these results solved how ELAC2 can recognize structurally different tRNAs in the nucleus and mitochondria: It can recognize nuclear and canonical mt-tRNAs by itself, but forms a multi-subunit complex with accessory proteins in mitochondria to process non-canonical tRNAs. (hh)